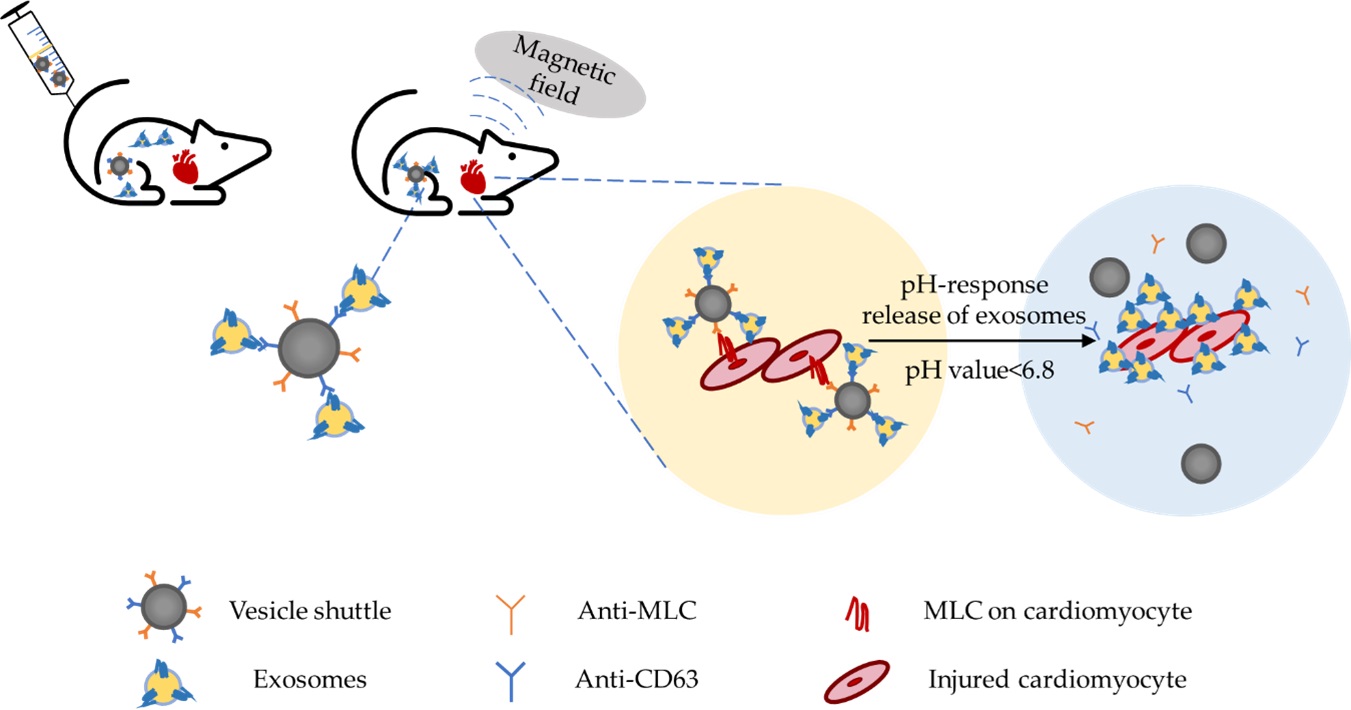
Downloads
Download


This work is licensed under a Creative Commons Attribution 4.0 International License.
Review
Nanoparticle-based Drug Delivery System for Post Myocardial Infarction Management
Minxuan Liu, Chiara Ramponi, Xiaoxue Fan, Xinzhuang Zhang, Liang Cao, Zhenzhong Wang, and Wei Xiao *
State Key Laboratory of New-tech for Chinese Medicine Pharmaceutical Process, Jiangsu Kanion Pharmaceutical Co., Ltd., Lianyungang, 222001, China.
* Correspondence: xw_kanion@163.com
Received: 15 October 2022
Accepted: 21 November 2022
Published: 21 December 2022
Abstract: Cardiovascular disease (CVD) is the leading cause of morbidity and mortality in the world. Myocardial infraction (MI) as one of the most harmful forms of ischaemic heart disease requires rigorous and tempestive approaches which are not met by current clinical interventions. Nanotechnology has developed promising clinical applications for imaging, diagnostic, gene delivery and tissue engineering, which makes this technology a potential candidate for novel therapeutic delivery approach. This review highlights several recent research reports regarding advances in drug delivery using nanoparticle-based (NP) strategies, as well as future challenges and opportunities.
Keywords:
myocardial infarction drug delivery nanoparticles1. Introduction
Cardiovascular disease (CVD) is the leading cause of morbidity and mortality in the world. Hearts damaged from CVD ultimately progress to heart failure (HF), a condition where the heart is unable to maintain a healthy cardiac output. The high morbidity and mortality of HF has recently become a burden in both developed and underdeveloped countries [1]. Myocardial infraction (MI) is one of the most harmful forms of ischaemic heart disease associated with HF. MI is characterised by cardiomyocyte loss and impaired cardiac performance [2]. Therapeutic strategies aimed at attenuating cardiomyocyte loss and preventing cardiac remodeling of the infract zone have been developed over the past decades. The delivery of thrombolytic agents, percutaneous coronary intervention, and bypass surgery are some of the most common clinical interventions [3]. However, such invasive surgical interventions have several limitations associated with the risk of reperfusion injury and bleeding [4]. The short half-life and the lack of specificity of pharmacological therapies, such as thrombolytic agents, also represents a limitation of current clinical protocols [5]. Stem cell therapy has been widely studied and developed to manage tissue regeneration in order to cope with the metastasis after MI. However, cell transplantation still faces the problem of low biocompatibility and limited cell behaviour [6].
The recent advances in the medical nanotechnology field have led to the development of novel diagnostics therapeutic platforms. For instance, nanoparticles (NPs) have promising clinical applications for imaging, diagnostic, gene delivery and tissue engineering [7]. Using NPs to deliver pharmacological treatments could potentially increase target specificity, improve the modulation of drug release hence reducing possible side effects. Moreover, the encapsulation or conjunction of therapeutic agents with NPs can enhance the compound solubility, reduce toxicity as well as improving its chemical stability in the circulation [8]. Surface modifications of NPs with peptides, antibodies or small molecules could further optimise the targeted delivery and biodistribution of therapeutic molecules [9]. Moreover, NPs have applications in the stem cell therapy where they have been shown to improve the biocompatibility of stem cells after transplantation [6].
Several types of NPs have been developed such as lipid-based NPs, polymeric NPs, micelles, inorganic NPs, and exosomes [9]. Inorganic magnetic NPs, made of metal compounds, carbon or silica, have raised great interest in the medical community. Magnetic NPs are usually made of iron oxide. The magnetic properties of these NPs can be exploited for both drug delivery and diagnostic tools. Magnetic NPs can interact with external magnetic fields, which could have attractive applications for diagnostic tools such as the magnetic resonance imaging (MRI) [10]. Moreover, the magnetism of these NPs also makes them powerful tool for magnetic resonance imaging (MRI) [11].
In this review, we presented several novel NP-based therapeutic delivery systems and discussed the recent advances of this promising field of modern medicine.
2. Enzyme-responsive Nanoparticle
The diameter of NPs ranges from 6 to 200 nm which makes them suitable for systemic transport. In addition, such small diameter makes them an ideal delivery system for minimally invasive injections. However, passive delivery still lacks specific target to the infract zones [12]. One of the issues associated with NP-based delivery systems is the rapid tissue clearance. For instance, this could become problematic in the treatment of post-MI left ventricle remodeling as the drug retention time might not be sufficient [13]. In order to improve the spatial specificity of the NPs to the infract zone and extend NP retention time, researchers developed an enzyme-responsive NP made of brush peptide and polymer amphiphiles (PPAs) [14]. The peptide has a specific sequence which is responsive to matrix metalloproteinases (MMPs). MMP-2 and MMP-9 are upregulated and overexpressed in the infract zone. Therefore, such enzyme-responsive NPs could reliably target the infract zones and improve the bioavailability of the therapeutic compound. When NPs interact with local MMPs, a morphological change is initiated, converting spherical nanoscale micelles into microscale, network-like materials. These morphological changes greatly enhance NPs retention in the infract area, with the compound being retained up to 28 days.
Prompt therapeutic intervention is essential in minimising the adverse consequences of MI on left ventricular (LV) remodeling. However, therapeutic agents are currently delivered by intramyocardial injection which could damage the thin walls of the infract area. The newly developed enzyme-responsive NP is an ideal delivery system for intravenous injection as it can avoid the risk of ventricular rupture. The ability of these spherical nanoscale micelles to mutate morphology has great translational value and it is a significant advance in the field of NP-based therapeutics.
3. Magnetic Antibody-linked Nanomatchmaker
Compared to other nanocarriers, iron oxide nanoparticles (IONPs) are relatively stable, biocompatible an environmentally safe [15]. These features make them more suitable for certain medical applications, such as contrast agents in MRI [11] and drug delivery [10]. However, cell toxicity and oxidation could potentially be an issue when using IONPs as a drug carrier. With the involvement of the coating of some organic and inorganic materials, iron-based NPs can be protected from environmental stimuli [16].
Stem cell therapy has been widely studied for its promising applications in tissue regeneration engineering, however, the complex interaction between therapeutic cells and injured tissue renders the application of such technology very challenging [17]. Antibody therapy have been approved by the Food and Drug Administration (FDA). Therefore, researchers developed a magnetic bifunctional cell engager (MagBICE) by conjugating anti-CD45 and anti-MLC antibodies to Ferumoxytol (trade name: Feraheme (FH)) [18]. This magnetic antibody-linked nanomatchmaker facilitates the interaction between the antibody and the antigen in therapeutic stem cells. After intravenous injection, MagBICEs capture endogenous CD34-positive cells during blood infusion and then specifically binds to the injured myocardium. This process is facilitated by anti-MLC antibodies. The intravenous infusion of MagBICE and BMCs not only reduces scar mass and size in the infarct zone, but also preserves wall thickness and promotes angiogenesis. It has been observed that MagBICE-BMC treatment preserves cardiac function and increases cell viability in the injured heart [18].
The iron core of these compounds allows MagBICE delivery and retention to be monitored by MRI. MagBICE targeting is specific to the heart with little off target effects on the lungs. A similar targeting specificity outcome has been observed between infarcted and non-infarcted areas. This was observed by analysing MagBICE biodistribution using MRI [18]. Although the use of MagBICE alone has therapeutic benefits, when used in combination with magnetic fields, its therapeutic value dramatically increases.
There are still limitations in this stem cell delivery system such as MRI image distortion and insufficient therapeutic stem cells to capture in blood circulation. Because of the wide clinical use of both ferumoxytol and recombinant human antibodies, these magnetic nanomatchmaker may easily be translated to clinical practice.
Exosomes are signal carriers mediating systemic information exchange and long-distance cell interactions, also known as nanoscale extracellular vesicles (EVs) [19]. Through transfer of membrane proteins, mRNAs, microRNAs and other molecules, exosomes have rather crucial effect on tissue homeostasis maintenance and pathological process [20]. According to previous studies on exosome modulation, it has been shown that exosomes may contribute to restoring cardiac function following MI. Therefore, exosome biodistribution manipulation can be a guilder of therapeutic delivery in MI management [17].
Jin and colleagues designed an inorganic-based nanoparticle ‘vesicle shuttle’ specialized in accurate targeting and selective release of ‘vesicle shuttle’ by combining the magnetic field technology and the microenvironment pH, as shown in Figure 1 [19]. This vesicle shuttle consists of an iron core, a silica (SiO2) shell and a poly (ethylene glycol) (PEG) corona. The iron core functions for the magnetic-field-induced targeting, and the SiO2 shell not only protects the metallic core from acid corrosion but also creates a proper base for the corona modification. After the vesicle shuttles are injected intravenously into the MI model, exosomes will be captured by anti-CD63 conjugated to the nanoparticle corona in the blood circulation. The other antibody conjugated to the corona is the anti-MLC, which plays a crucial role in precisely targeting the infract area. This is facilitated by the local magnetic field for physical enrichment of the vesicle shuttles. Infract areas are characterised by acidosis with pH values lower than 6.8. Acidic pH induces the cleavage of hydrazone bonds and shedding of the corona, resulting in exosome release.
Figure 1. Schematic diagram of the vesicle shuttle delivery system. The anti-CD63 antibodies conjugated to the vesicle shuttle capture and attach to endogenous circulating exosomes. Dual targeting of this strategy using both an external magnet, which attracts the magnetic vesicle shuttle to the infarct area, and a conjugated anti-MLC antibody, which targets MLC on damaged cardiomyocytes. Release of the exosomes triggered by the infarct environment of pH < 6.8, acidosis-induced cleavage of hydrazone bonds and shedding the corona leads to the selective release of exosomes.
Although CD63 is capable of capturing exosomes, it is expressed in EVs [21]. Therefore, increased therapeutic benefits could be achieved by designing unique antibody markers. The concept of in vivo manipulation of exosome biodistribution can be extended to other disease management. Overall, this vesicle shuttle still needs further investigation, because different pathological microenvironments could have different effects on transferred exosomes and NPs functions.
4. EV Therapy Combined Nanoparticle
While EV signal transfer offers promising drug delivery system, it remains unclear how this endogenous biodistribution is regulated [19]. It is crucial to further investigate how systemic distribution, uptake and excretion of EVs can be monitored. Moreover, a novel strategy to encapsulate superparamagnetic iron oxide (SPIO) NPs into EVs have been developed,which results in an optimised MRI detection [22]. Such mechanism has not been reported in the literature before. Studies on in vivo MRI-based EV are limited by the low sensitivity of MRI and SPIO nanoparticles aggregation [23]. This research has highlighted on optimized electroporation with high efficiency of labelling and purification. The surface modulation of SPIO nanoparticles was achieved through conjugation with hexa-histidine peptides. These SPIO nanoparticles bound to His-tag can selectively bind to Ni2+ immobilized nitrilotriacetic acid (NTA) agarose resins (Ni-NTA) columns. This contributes to the high purification efficiency of unloaded free SPIO-His after encapsulation (up to 97.4%). SPIOs labelled successfully and ensure an adequate sensitivity for MRI detection, with the high removal rate of free SPIO-His, a sufficient specificity of MRI detection can be achieved without interferences from free NPs.
This research takes iPSC-derived EVs as a functional therapeutic EVs with no nuclei. Therefore, they can be applied clinically without any concern for genetic instability and tumorigenicity. The therapeutic EVs can be individualized from autologous iPSCs of the same patients, which also avoids immunogenicity and ethical concerns. This technology is capable of in vivo quantitative assessment of spatial-temporal distribution of magnetically labelled EVs in the system. Both maximized encapsulation rate and convenient purification approaches contribute to a good balance of purification against yield. This generates a powerful tool for large scale applications in the future.
5. Challenges and Opportunities
With the development of nanotechnology and its combination with therapeutic delivery system, researchers have overcome several limitations related to the bioavailability and the biocompatibility of drug delivery in CVDs. MI requires rather rigorous and tempestive approaches which are not met by current clinical interventions. Current pharmacotherapy exhibits low absorption, rapid excretion, and toxicity if administrated at high dose. However, with the encapsulation of NPs and NP surface modification, these issues could be solved readily. Traditional Chinese Medications are also characterised by low solubility and bioavailability. Although the safety aspects and the effects of NPs at different disease stages need further investigation, magnetic NPs and stem cell regeneration therapy have been widely used clinically. This could perhaps suggest that NP-based stem cell therapy will translate to clinical practice in the near future. Moreover, several Chinese herbal medicines (TCM) have been shown to improve cardiac function after MI [24,25]. NP-based delivery system can be a novel option for researchers to study TCM and perhaps have positive clinical effects in the management of MI and CVDs in the future.
Author Contributions: Writing—original draft preparation, Minxuan Liu, Xiaoxue Fan; writing—review and editing, Chiara Ramponi, Minxuan Liu; supervision, Xinzhuang Zhang, Liang Cao; project administration, Zhenzhong Wang, Wei Xiao. All authors have read and agreed to the published version of the manuscript.
Funding: This research received no external funding.
Conflicts of Interest: The authors declare no conflict of interest.
References
- Nichols M.; Townsend N.; Scarborough P.; et al. Cardiovascular disease in Europe 2014: epidemiological update. Eur. Heart J., 2014, 35(42): 2950-2959. DOI: https://doi.org/10.1093/eurheartj/ehu299
- Reed G.W.; Rossi J.E.; Cannon C.P. Acute myocardial infarction. Lancet, 2017, 389(10065): 197-210. DOI: https://doi.org/10.1016/S0140-6736(16)30677-8
- Marín-Juez R.; El-Sammak H.; Helker C.S.M.; et al. Coronary revascularization during heart regeneration is regulated by epicardial and endocardial cues and forms a scaffold for cardiomyocyte repopulation. Dev. Cell, 2019, 51(4): 503-515.e4. DOI: https://doi.org/10.1016/j.devcel.2019.10.019
- Heusch G. Myocardial ischaemia-reperfusion injury and cardioprotection in perspective. Nat. Rev. Cardiol., 2020, 17(12): 773-789.doi:10.1038/s41569-020-0403-y. DOI: https://doi.org/10.1038/s41569-020-0403-y
- Yang L.Q.; Peng J.J.; Shi A.P.; et al. Myocardium-targeted micelle nanomedicine that salvages the heart from ischemia/reperfusion injury. ACS Appl. Mater. Interfaces, 2022, 14(34): 38562-38574. DOI: https://doi.org/10.1021/acsami.2c11117
- Chen J.L.; Huang N.; Ma B.L.; et al. Guidance of stem cells to a target destination in vivo by magnetic nanoparticles in a magnetic field. ACS Appl. Mater. Interfaces. 2013, 5(13): 5976-5985. DOI: https://doi.org/10.1021/am400249n
- Bahadur S.; Sachan N.; Harwansh R.K.; et al. Nanoparticlized system: promising approach for the management of alzheimer’s disease through intranasal delivery. Curr. Pharm. Des., 2020, 26(12): 1331-1344. DOI: https://doi.org/10.2174/1381612826666200311131658
- Pala R.; Anju V.T.; Dyavaiah M.; et al. Nanoparticle-mediated drug delivery for the treatment of cardiovascular diseases. Int. J. Nanomed., 2020, 15: 3741-3769. DOI: https://doi.org/10.2147/IJN.S250872
- Pan Q.; Xu J.; Wen C.J.; et al. Nanoparticles: promising tools for the treatment and prevention of myocardial infarction. Int. J. Nanomed., 2021, 16: 6719-6747. DOI: https://doi.org/10.2147/IJN.S328723
- Saikia C.; Das M.K.; Ramteke A.; et al. Effect of crosslinker on drug delivery properties of curcumin loaded starch coated iron oxide nanoparticles. Int. J. Biol. Macromol., 2016, 93(Pt A): 1121-1132. DOI: https://doi.org/10.1016/j.ijbiomac.2016.09.043
- Malekzadeh A.M.; Ramazani A.; Rezaei S.J.T.; et al. Design and construction of multifunctional hyperbranched polymers coated magnetite nanoparticles for both targeting magnetic resonance imaging and cancer therapy. J. Colloid Interface Sci., 2017, 490: 64-73. DOI: https://doi.org/10.1016/j.jcis.2016.11.014
- Prabhakar U.; Maeda H.; Jain R.K.; et al. Challenges and key considerations of the enhanced permeability and retention effect for nanomedicine drug delivery in oncology. Cancer Res., 2013, 73(8): 2412-2417. DOI: https://doi.org/10.1158/0008-5472.CAN-12-4561
- Suarez S.; Almutairi A.; Christman K.L. Micro- and nanoparticles for treating cardiovascular disease. Biomater. Sci., 2015, 3(4): 564-580. DOI: https://doi.org/10.1039/C4BM00441H
- Nguyen M.M.; Carlini A.S.; Chien M.P.; et al. Enzyme-responsive nanoparticles for targeted accumulation and prolonged retention in heart tissue after myocardial infarction. Adv. Mater., 2015, 27(37): 5547-5552. DOI: https://doi.org/10.1002/adma.201502003
- Lu A.H.; Salabas E.L.; Schüth F. Magnetic nanoparticles: synthesis, protection, functionalization, and application. Angew. Chem. Int. Ed., 2007, 46(8): 1222-1244. DOI: https://doi.org/10.1002/anie.200602866
- Arias L.S.; Pessan J.P.; Vieira A.P.M.; et al. Iron oxide nanoparticles for biomedical applications: a perspective on synthesis, drugs, antimicrobial activity, and toxicity. Antibiotics, 2018, 7(2): 46. DOI: https://doi.org/10.3390/antibiotics7020046
- Cheng M.; Yang J.J.; Zhao X.Q.; et al. Circulating myocardial microRNAs from infarcted hearts are carried in exosomes and mobilise bone marrow progenitor cells. Nat. Commun., 2019, 10(1): 959. DOI: https://doi.org/10.1038/s41467-019-08895-7
- Cheng K.; Shen D.L.; Hensley M.T.; et al. Magnetic antibody-linked nanomatchmakers for therapeutic cell targeting. Nat. Commun., 2014, 5: 4880. DOI: https://doi.org/10.1038/ncomms5880
- Liu S., Chen X. , Bao L.;et al., Treatment of infarcted heart tissue via the capture and local delivery of circulating exosomes through antibody-conjugated magnetic nanoparticles. Nat. Biomed. Eng., 2020 4(11) 1063-1075. DOI: https://doi.org/10.1038/s41551-020-00637-1
- Théry C.; Zitvogel L.; Amigorena S. Exosomes: composition, biogenesis and function. Nat. Rev. Immunol., 2002, 2(8): 569-579. DOI: https://doi.org/10.1038/nri855
- Kowal J., Arras G., Colombo M.; et al., Proteomic comparison defines novel markers to characterize heterogeneous populations of extracellular vesicle subtypes. Proc. Natl. Acad. Sci. USA, 113 (2016) E968-77. DOI: https://doi.org/10.1073/pnas.1521230113
- Han Z.; Liu S.Q.; Pei Y.G.; et al. Highly efficient magnetic labelling allows MRI tracking of the homing of stem cell-derived extracellular vesicles following systemic delivery. J. Extracell. Vesicles, 2021, 10(3): e12054. DOI: https://doi.org/10.1002/jev2.12054
- Busato A.; Bonafede R.; Bontempi P.; et al. Magnetic resonance imaging of ultrasmall superparamagnetic iron oxide-labeled exosomes from stem cells: a new method to obtain labeled exosomes. Int. J. Nanomed., 2016, 11: 2481-2490. DOI: https://doi.org/10.2147/IJN.S104152
- Li H.; Zhu J.; Xu Y.W.; et al. Notoginsenoside R1-loaded mesoporous silica nanoparticles targeting the site of injury through inflammatory cells improves heart repair after myocardial infarction. Redox. Biol., 2022, 54: 102384. DOI: https://doi.org/10.1016/j.redox.2022.102384
- Wu X.X.; Liu L.; Zheng Q.L.; et al. Protocatechuic aldehyde protects cardiomycoytes against ischemic injury via regulation of nuclear pyruvate kinase M2. Acta Pharm. Sin. B, 2021, 11(11): 3553-3566. DOI: https://doi.org/10.1016/j.apsb.2021.03.021